Today, the majority of biologic drugs, including mRNA vaccines, are made in bioreactors. These glass or stainless steel vessels create a controlled environment for bacteria, yeast, insect, or Chinese hamster ovary cell lines to grow and divide. The process churns out nucleic acids, enzymes, antibodies and other recombinant proteins on a large scale. It’s the main, but not exclusive, way to produce biomolecules at scale.
A small group of biochemists have shown that plants could produce the same types of proteins. Some argue they do it better.
The process goes by many names — plant molecular farming, biopharming, or plant molecular pharming. These biochemists believe such plants are ripe for recognition.
It’s not uncommon for a pharmaceutical technology to sit on the backburner until the right opportunity emerges for it to burst into the mainstream. mRNA science is a shining example. The idea of mRNA vaccines had kicked around for three decades before the famous COVID-19 jabs arrived. By COVID times, mRNA technology was already well-known and well-understood; the problem was that there was no place to produce it on a grand scale. The science sat on the back burner until the right opportunity emerged for mRNA to burst into the mainstream.
Propelled by the federal government’s push to create a vaccine against SARS-CoV-2 as quickly as possible, Pfizer and Moderna both scaled up rapidly. To do so, Pfizer built specific modular clean room pods, which didn’t exist until 2009. Teams assembled prefabricated glass cubicles off-site and custom-fitted them into a large factory. Pfizer received as many sterile partitions as their project required. The company erected their first two fully functioning mRNA vaccine facilities in less than a year.
With all hands on deck, pharma researchers laid the groundwork to develop and test the first mRNA vaccine, which was authorized for emergency use by the Food and Drug Administration less than a year after the virus was first sequenced — a record.
mRNA’s long-awaited breakthrough didn’t fall out of some epiphany in mRNA science, it came when the right context demanded it.
But for biopharming to truly take off in these scenarios, the niche group of biochemists championing PMPs may need to temper the hopes they’ve had for the technology. Just one PMP has been approved by the Food and Drug Administration. Another, a COVID vaccine, was approved by Canadian health authorities. Few proponents of biopharming believe plant-made pharmaceuticals, or PMPs, are poised to outright replace the industrial systems pharmaceutical companies have already invested in. Instead, PMPs could shine as small-batch medicine — personalized therapies, orphan drugs, and vaccines, and drugs that treat rare diseases.
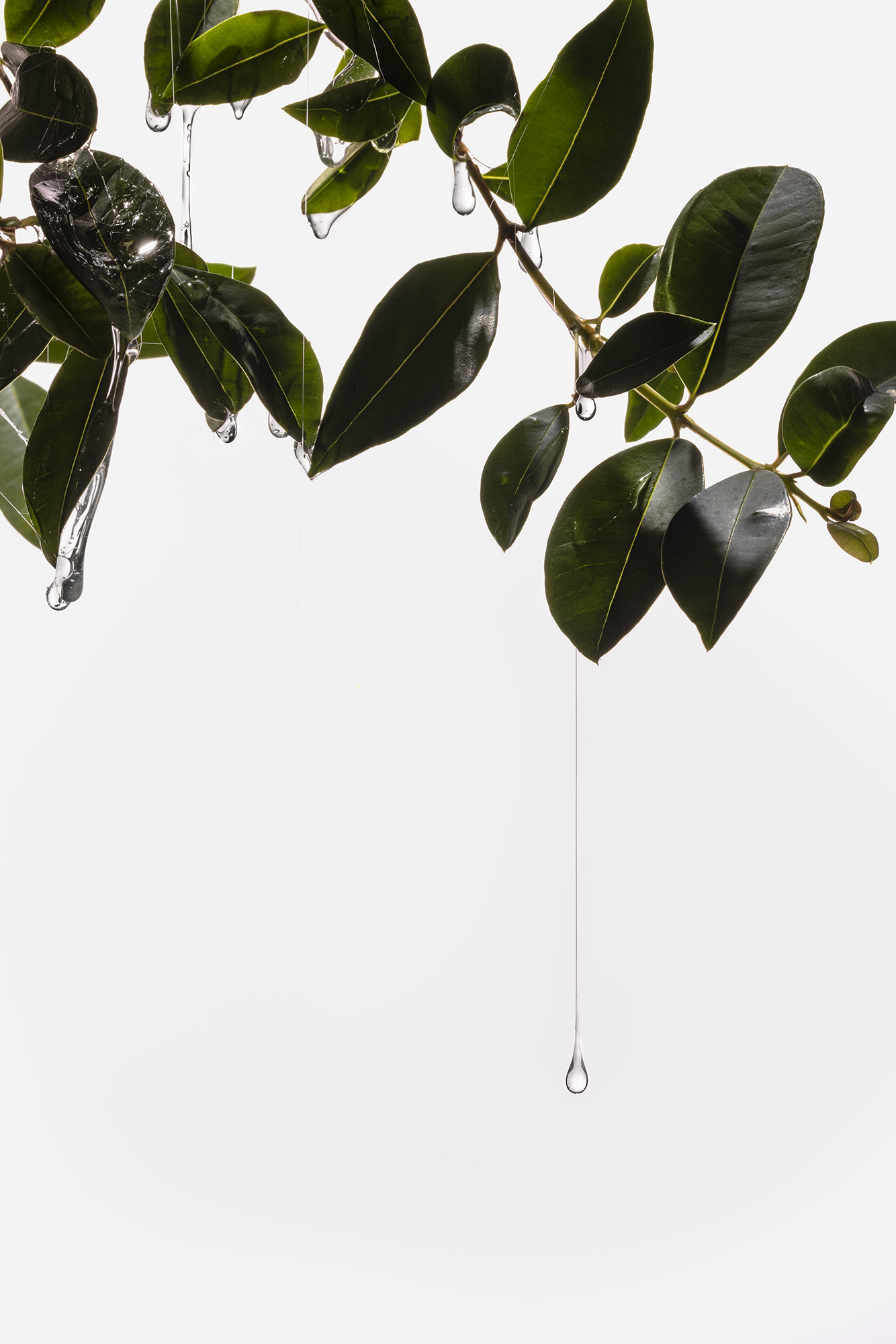
Living Bioreactors
A seed doesn’t need much to morph into complex life. Take Nicotiana tabacum, the tobacco plant, for example. When smothered in damp soil, cocoa-colored tobacco seeds absorb moisture and oxygen through a protective coating on their outermost layer. Within 10 days, delicate roots propel the plant’s first two leaves upwards in search of sunlight. Nicotiana tabacum cells can multiply 100-fold every week, each churning out calculated molecules called secondary metabolites.
The entire plant kingdom produces at least 200,000 of these chemical compounds crucial to plant survival across the globe, like the secondary metabolites that serve as natural antifreeze in carrots and winter rye when cold weather hits. Others defend against pathogens or the gnawing gobs of herbivores. “Plants are great chemists,” says George Lomonossoff, a virologist at the John Innes Center in Norwich, U.K. “You can also make plants make a lot of things they normally wouldn’t.”
With some coaxing, plants can produce tailor-made proteins, enzymes, antigens and antibodies as they would other cellular products. This fact has drawn scientists like Lomonossoff to plants in search of new ways to make biologic drugs for humans. There are two ways in which scientists can genetically modify a plant to create metabolites that humans can use, and the most commonly championed such biopharming tactic is “transient expression.”
The end products of transient expression don’t include any plant material at all, only the viral or human proteins the plant was programmed to make. Biologists insert foreign DNA into a plant cell and that “transforms” the plant’s metabolic offerings. But those instructions don’t pass down to the next generation of plants. Transient expression is, after all, transient.
When crops mature in indoor greenhouses (for tobacco plants that takes about six weeks), they’re drawn a bath and a soil bacterium known as Agrobacterium tumefaciens takes over.
The bacterial bath is infused with fragments of proteins and viral genetic material that can elicit an army of antibodies that can be the active ingredients in human drugs. Then, the bath becomes a vacuum chamber. Trapped air rushes out of the plant’s intracellular spaces. The biological slurry floods in. In just a few moments, the plant’s biomass amplifies to roughly 1,000 times what it was before. Agrobacterium tumefaciens transfers the foreign DNA to the plant cells, and almost immediately, each modified cell begins to produce designer proteins.
After six weeks of initial growth, it takes the plants less than a week to make the proteins. Biologists then blitz the plants in a giant blender. The resulting vat of green slime contains millions of recombinant proteins. These can be used to create everything from personalized cancer vaccines to lateral flow tests. The process doesn’t change the genome that the plant stores in its seeds. It’s one-and-done. The entire two month process is relatively short when it comes to drug manufacturing.
However, this process is not without the usual slow-downs of manufacturing biologics from regulation to teasing out the best drug delivery method. Although growing tobacco plants is cheap and relatively fast, the green slime has to be purified.
When a plant is altered to make a foreign protein, it’s still also making other metabolites — the ones it actually needs to survive. Transient expression molecules are primarily destined for injectable vaccines or drugs. These require extremely pure versions of these antibodies, proteins and enzymes. Everything that isn’t a tailor-made recombinant protein has to be filtered out. The same separation is necessary with medicinal molecules and the unusable side products produced from mammalian and yeast cell cultures made in bioreactors. Even if the drug isn’t designed to be injected, the tobacco plants common in biopharming produce nicotine and other molecules that can’t be mixed into drugs, so those have to go. In any case, the downstream purification process is both costly and tedious.
Biopharming proponents often blame a lack of clear regulation for PMPs as the deciding factor keeping these particles from going mainstream. Drugs made in bioreactors have far clearer standards. Henry Daniell, a biochemist and vice chair of the Department of Basic & Translational Sciences at the University of Pennsylvania, disagrees that regulation is the biggest hurdle keeping PMPs from the mainstream.
“It’s not how you make it, it’s how you deliver it that makes it costly,” he says. “Without being more affordable, it’s not competitive.”
Drug development is too crowded a field to get by without a cost advantage. But the limits of transient transformation often make it as expensive on the backend as the same molecules produced in yeast, bacteria, insect or hamster cells.
Daniell isn’t against growing medicinal proteins in tobacco plants, but he argues the end product doesn’t make drugs stand out compared to pharmaceuticals made from CHO, bacteria or yeast cells. “Once you purify it, you lose a lot of the proteins and you have to put it in cold transportation storage. It has to be injected,” he says. “All that makes transient expression less competitive.”
Fixed Genes
Biopharming’s focus on transient expression may be one of its biggest hitches. Daniell is in a camp of scientists who believe the answer could lie in making recombinant proteins with another approach, known as “stable transformation,” with plants you’d find in your grocery store’s produce aisle.
Consider lettuce. A head of lettuce grows in four to six weeks, depending on the variety. Using a contraption called a gene gun (which looks a bit like the device chain jewelry stores use for ear piercing) scientists blast bits of viral or human DNA into the cells of lettuce leaves. The foreign genes are encased in tiny flecks of gold or tungsten and hitch a ride on high pressure helium gas. Once the genetic material pierces the cell walls, and settles into a permanent space within the plant’s chromosomes, any offspring the lettuce has will also house this DNA that includes biopharming instructions.
Because stable translation utilizes common edible plants, it lends itself well to edible therapies that don’t require pure forms of the introduced genetic material, like non-injectables. The final product isn’t filtered. It includes all the plant material as well as the foreign genes. Scientists have their eye on stable translation to make edible vaccines, though none have thus far been approved for human use. The altered plant matter could also lend itself to creating shelf-stable alternatives to existing drugs.
Daniell is working to develop an edible insulin at a fraction of the cost of the injectable version. He and his colleagues used a gene gun to integrate insulin genes into lettuce leaves. The leaves were then freeze dried, ground into a powder, and slipped into capsules. So far, the insulin has proven to be as effective in mice as the insulin made in bioreactors. It also doesn’t need to be refrigerated and can forgo the extensive purification process since the vast majority of humans can ingest lettuce without issue. And since it wouldn’t be injected, an edible insulin like this wouldn’t have the added cost of purification.
These merits still may not be enough to quell the concerns of Big Pharma — or the public.
Historically, plans to grow biopharming flora outdoors has led people to lump the practice in with controversial GMO crops and worry about mutated plants unwittingly spreading genes to nearby flora.
This actually happened in Nebraska during the summer of 2002. A biotech company called ProdiGene was testing the feasibility of coaxing a crop of genetically modified corn to produce a diagnostic protein called avidin. They harvested the pharma crop, and prepared the land to house neat rows of soybeans destined for infant formula and veggie burgers. When a combine severed and collected the bean plants, a few of ProdiGene’s modified kernels got swept up, too. The United States Department of Agriculture detected the foreign material in what was supposed to be pure soybeans. 500,000 bushels had to be destroyed.
It’s understandable that people would not want stray pharmaceutical proteins in their veggie burgers. And some executives fear that the switch to molecular farming could elicit pushback from people concerned with food safety. (This, in turn, tips the scales toward transient expression, which doesn’t carry the risk of cross-contamination.) However, proponents argue the risk for cross-contamination can be mitigated in two ways. The first is that molecular farming is almost exclusively done in indoor greenhouses rather than in outdoor fields. The second lies in a plant’s biology.
Plants have three genomes: one in the nucleus, a second in chloroplast, and the third in the mitochondria. When a gene gun blasts a plant cell, the new genetic code sits in the chloroplast. Both the chloroplast and mitochondrial genomes are inherited through the maternal line. Only the nuclear genome trafficks code from a male plant. And only this genetic material can drift into another plant in a cross-pollination event that spreads modified genes to another plant.
“If it is in the chloroplast there is no way the gene can escape,” Daniell says. However, he added, a flowering modified plant can release seeds that germinate with the bits of foreign programming. Not allowing the plant to go to seed before it’s harvested can ensure this doesn’t happen.
Losing the Arms Race
Even after 30 years of research and development, only a handful of PMPs have been okayed for human use. None have been touted as major success stories to the likes of mRNA vaccines.
One reason is that, so far, there hasn’t been a time in which a PMP hits the market before a similar drug made in the traditional way has already become available. In 2012, the drug Elelyso became the first PMP to gain FDA approval. Israeli biotech company Protalix Biotherapeutics grew the novel enzyme replacement drug in modified carrot plants to target a rare genetic condition called Gaucher disease, which causes lipids to build up in the organs and bones. Worldwide, it impacts 1 in 40,000 people.
The FDA fast-tracked Elelyso’s approval process after Genzyme’s competing product, Cerezyme, suffered from production issues. The PMP also cost about 25% less than its competition: around $1,000 per injection, or $150,000 per year. (Cerezyme’s out-of-pocket cost was around $200,000 in 2012.) A drug shortage combined with a high drug price created a unique environment for Elelyso to launch into 23 markets around the world. However, the PMP still didn’t become more popular than Cerezyme once the production issues were resolved. And although doctors still prescribe the drug today, Elelyso did not pave the way for a windfall of new PMPs.
Around eight years later, a vaccine made from transient expression in tobacco plants met a harsher fate. In December 2021, Canadian scientists were releasing the results of a Phase 3 clinical trial for a particularly intriguing COVID vaccine that appeared to be a potent pandemic tool, even against the alphabet soup of emerging variants.
The vaccine’s maker, Canadian biotech company Medicago, cued tobacco plant cells to create “viruslike particles” that mimicked COVID’s unique spike protein. The process required much less space than traditional means of making vaccines. It produced failures quickly, which streamlined the traditionally arduous research and development process. Less than 24 months from the project’s inception, the end products were precise and offered something no other COVID vaccine could at the time: its ability to be stored at room temperature. Most COVID vaccines required special freezers for transport and storage, which precipitated an enormous inequity in global public health. Thermostability was a truly huge factor.
Then, before obtaining approval in Canada, the project was killed by Mitsubishi, which bought the company Medicago that created the vaccine. Many speculate it was because the company didn’t see the vaccine as profitable enough compared to the existing COVID vaccines, despite its thermostability.
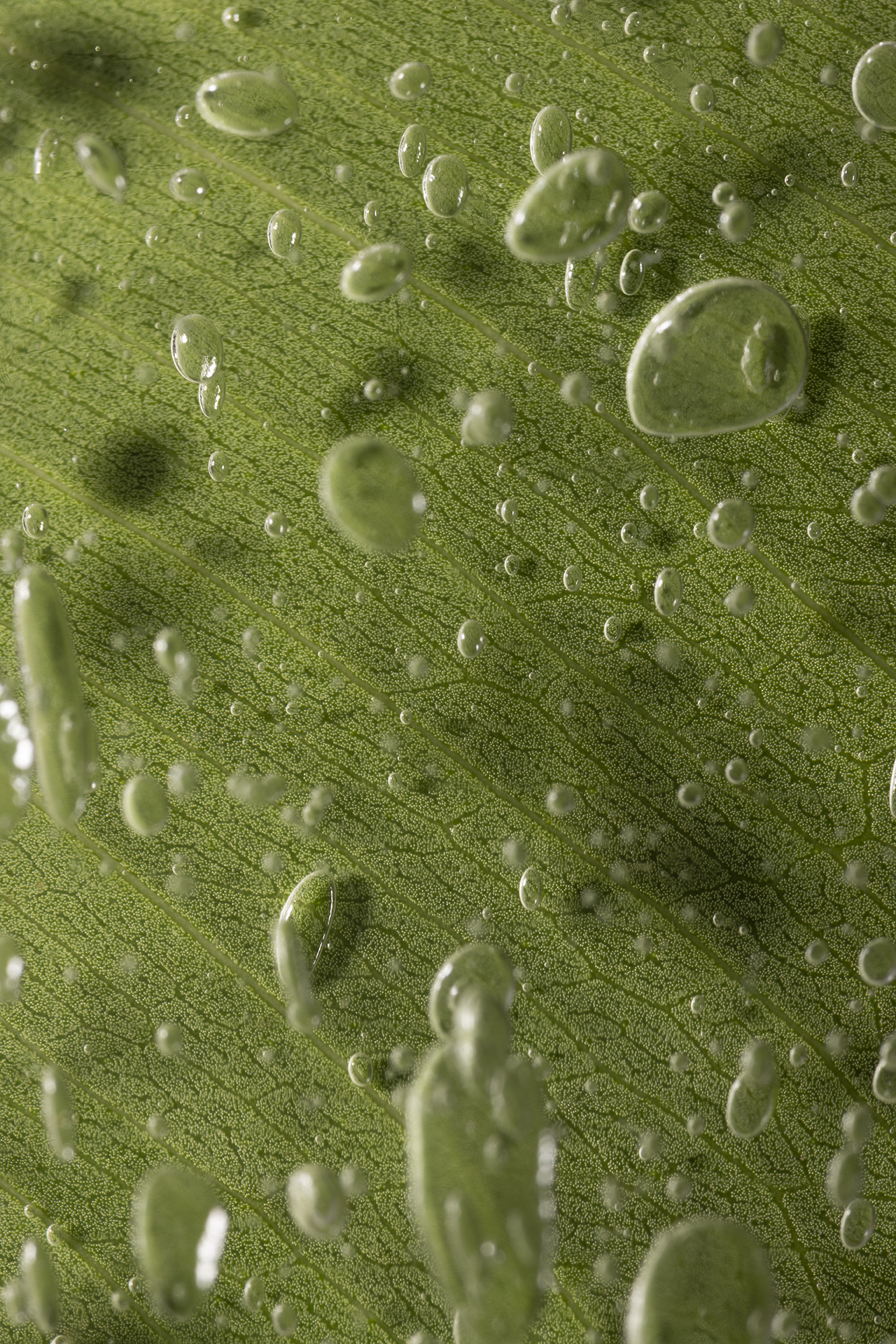
Scalable Precision
In the coming years, the $12 trillion global healthcare industry will witness a revolution of precision medicine and a wave of novel fungi, viruses, and bacteria that cause human diseases. Plant-made pharmaceuticals would lend themselves to both.
Large pharmaceutical companies have already bet big on large factories lined with steel bioreactors full of yeast or insect cells. Making the switch to molecular farming –– whether that be transient or stable expression –– would mean deviating from this infrastructure in favor of a plant-based technology with unique risks of public acceptance and regulatory approval.
“It’s easy to scale, but you’re in competition with everyone who uses existing bioreactors. They have a vested interest in not changing their business model,” says Ed Rybick, director of the URC Biopharming Research Unit at the University of Cape Town in South Africa. “The brash young startups are the only ones who have been successful.”
What might change the calculus is, as with mRNA, the right medical and economic context. The breakthrough moment may come from an eager start-up and it will most likely address a growing demand for precision medicine.
PMPs will not replace proteins, enzymes and antibodies created in the traditional way because companies have already invested huge amounts of money into bioreactors and the infrastructure to make drugs from bacteria, yeast, and animal cells; but a changing landscape could open up a niche that would make the practice attractive to pharmaceutical companies.
As drugs become more personalized, they treat fewer people, which means companies don’t need the huge reactors they currently use. PMPs, on the other hand, can easily scale up or down to produce molecules that more precisely meet patients’ needs. For instance, researchers are already working to create personalized oncological vaccines that teach a person’s own immune system to seek out, attack, and kill its unique cancer cells. Using molecular farming, these vaccines could be developed and manufactured within a couple of weeks.
Because crops are so easy to scale, each person could have a portion of a plot dedicated to their own vaccine –– Joe’s vaccines in these 100 plants, Maria’s in these others.
Without clear regulatory framework, it’s not yet clear how approval for such drugs might play out. It’s true that Medicago’s COVID vaccine was approved for use in Canada, and Elesyo in the US. But PMP regulations would need to consider how the PMP was created — transient or stable expression — and whether individualized medicines, such as Joe’s and Maria’s cancer vaccines, could advance under a blanket approval based on their protocol, or would need individualized approval, too.
The regulatory hurdles remain an open question, but technologically molecular farming has the ability to produce one thing in massive numbers, or to make just enough of many different things. This duality of scale is crucial. PMPs could have a big impact in the places that are currently underserved by existing drug manufacturing plants, including several countries in Africa and Southeast Asia.
According to Rybick, “There is the real possibility of using plant molecular farming in areas of the world with endemic diseases that are not seen in Europe or the U.S.”
He offers Lassa fever, as an example. The Lassa virus causes hemorrhagic fever in at least eight West African countries. It’s a huge burden on healthcare systems in Sierra Leone and Liberia, where as much as 15 percent of hospital admissions are for Lassa fever. An antiviral used to treat respiratory syncytial virus (RSV) has had some success in treating severe cases (about 20% of all infections) but it usually has to be combined with other drugs. Molecular farming could produce a pointed antiviral designed to target the Lassa virus, or even a vaccine to prevent it, Rybick says. Rybick’s lab sees Lassa as one of many potential futures for PMP in Africa. The lab is poised to make that future happen — with enough funding, Rybick hopes to make these drugs with the viral DNA his lab has banked.
The technology could also be a solution for inequitable access to drugs that already exist. Rituxan is the first monoclonal antibody therapy for non-Hodgkin’s Lymphoma. HIV remains a main driver of lymphoma cases around the globe, especially in Sub-Saharan Africa, but access to the critical drug is often far easier in the United States and Europe. A generic version of Rituxan exists, but making a biosimilar therapeutic locally could allow the cancer treatment to reach more of the patients who are most at risk of developing lymphoma.
No silver bullet will ever combine the best features of all drug-making methods and leave the worst by the wayside. The future must include different markets that utilize different methods depending on need.
“It’s important to not just rely on one thing, to add strings to your bow,” says Lomonossoff. “You never know what is around the corner and what will work best.”